2001 NSS Roadmap: Technological Barriers to Space Settlement
From Ad Astra January-February 2001
by Gordon R. Woodcock
Lack of Affordable Transportation to Space
It’s very costly today. Launching a pound of payload into space on the shuttle costs about $10,000; a typical expendable launch vehicle (ELV) charges about $5,000, and the cheapest ELV prices are about $3,000. If the shuttle were converted by putting a passenger cabin in the payload bay, it could carry about 50 passengers. At a launch cost of some $400 million, the ticket price would be at least $8 million. Only the wealthiest can afford such a price and few would choose to; at this price it is unlikely even one such excursion would ever get off the ground.
Pundits and technologists have been promising that low cost space transportation is “just around the corner” for at least forty years. Shuttle was supposed to be low cost. So what’s the story, really?
When we watch a shuttle launch, it appears a prodigious amount of energy is being used. So much that cost simply can’t come down a lot?
The energy is not really that great; it is just released very rapidly. The theoretical energy of an object in orbit is about 4 kilowatt hours per pound. At typical retail energy cost of 10¢ per kWh, the result is 40¢ per pound. So why are we paying $5,000/lb? Are we doing something fundamentally wrong? Probably, but we don’t know of a practical way to get to space except by rocket propulsion.
We know the cost of operating a mature-industry transportation system (like motor vehicles or air transport) is about four to five times the underlying cost of the energy used. Figure it for a typical car: the gas costs about 6¢ per mile and total cost to run a car is about 30¢ per mile.
Since we can’t have low cost by continuing to throw away expensive aerospace hardware on every launch, let’s consider the actual energy cost for reusable launch vehicles (RLVs). For a typical propellant load of hydrogen and oxygen, the cost is about $10/lb of payload. Applying 5 x energy cost as earlier, we expect $50/lb for a mature space transportation industry using RLVs. Evidently this isn’t a mature industry.
Why not? Because demand is too small compared to the large investments needed to develop an RLV and field a fleet of three or more vehicles. Paying off these investments means that RLVs are more cost-effective than ELVs only at traffic demand of 100 missions per year or more. Current worldwide demand is 20 to 40. The most comparable industry, commercial aviation, experiences demand of millions of flights per year. Calculations show that RLVs could approach the “mature industry” cost projection at a demand level of “only” thousands per year.
If demand should grow to such numbers, a successful RLV must have certain other attributes: Turnaround time on the ground one or two days, low probability of loss, and vehicle life of more than 1,000 total flights. Two graphs illustrate: the first shows effects of traffic demand and vehicle flight rate (the latter dictated by turnaround time), and the second shows potential for cost reduction with all attributes selected to define a “successful RLV.”
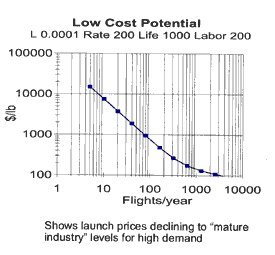
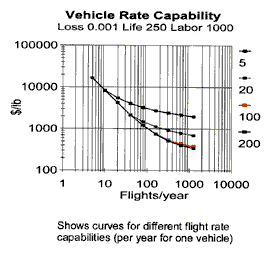
Without high demand, the RLV business case does not close no matter how good the RLV’s other attributes. Clearly, if space settlement were undertaken, traffic demand would be very large. However, without significant reduction in costs, a settlement program is seen as economically impossible. It’s a “Catch-22.”
Government-funded human exploration supported by RLVs would improve the business case, but probably not enough for private investment: A modest lunar base needs about 25 launches/year to support four lunar trips per year. Mars exploration, assuming one trip per Mars opportunity, needs about 50 per year to put up enough equipment and propellant, and support orbital assembly operations. Other NASA demands are about 10 per year, and commercial communications, up to 20 per year.
The projected total demand is about 100 launches/year, and the industry’s revenue at NASA’s target cost of $1,000/lb would be about $4 billion annually. That’s actually a little less revenue than today. Investors and company boards will ask, “Why take high business risk if revenue doesn’t grow even with success?”
What about commercial demand? For low prices to generate a lot of demand, we must find customers for which the price of transportation is their main cost.
A generation of studies has identified only people (i.e., tourists) as cheap “payloads” for which this is true. The “payload” represented by one tourist is on the order of 500 lbs., since accommodations such as a pressurized cabin, life support and a seat, count as payload as defined in the launch industry. At $1,000/lb launch cost, a price about $1 million per customer, I estimate a few thousand a year and roughly a billion total revenue. At $100/lb, $100,000 per ticket, I estimate millions of customers per year, and tens of billions revenue. Unfortunately, I have very low confidence in these estimates; there just isn’t enough supporting data. We have two data points: Dennis Tito was willing to pay $20 million for a ride to Mir, and it was reported a few years ago that John Denver offered NASA $10 million for a shuttle ride. (NASA declined.) We also know that many
customers pay high prices for rides in high performance Russian jet fighters, and that exotic-tour companies have collected sizable deposits on future space flights.
Can we assess or even test the tourism market? The usual systems engineering approach, highly favored by NASA, won’t work.
By “usual” I mean the method developed for defense projects where a “threat” is well-defined, and “requirements” to negate it are equally well-defined. In this case, requirements can’t be defined because there is no threat nor an identified set of customers to survey or sign up.
We need an opportunity and innovation approach, like the Model T Ford or the personal computer. Entrepreneurs had a concept of a market and a product to tap the market. They were able to finance building the products; they proved the market was there.
There are entrepreneurs who want to test the space tourist market. But financing is tough. They need help. They need an analog to the mail contracts of the 1920s that helped launch the aviation industry. Technology is also a challenge; they need technology help to move beyond suborbital rocket planes.
These entrepreneurs propose suborbital rocket planes which would fly to the “edge of space.” They would use current technology to reach more than 100 km altitude, capable of winning the X Prize. They would carry 3 to several passengers. The business case probably closes between $10,000 and $50,000 per ticket. However, it’s “high risk”; presently none of these entrepreneurs are fully funded.
If such a venture proved the market, the next step is tourists to orbit! The first orbital tour planes would probably carry 20 to 30 passengers, like the old DC-3. This would be a 15,000-lb-payload vehicle. To reduce risk, one would initially build the smallest RLV likely to repay its investment. At $1 million per ticket, I estimate 1,000 passengers per year, a flight a week at 20 passengers. Projected revenue of $1 billion supports $2 to $5 billion investment. This is feasible for this size airplane if suitable engines are available (today they are not).
Poor Safety Record for Launch Vehicles
This is the second barrier. Quite simply, it follows from the fact that launch vehicles evolved from military rockets, for which a reliability of 0.95 is good enough. A successful RLV, especially if it is to carry passengers, needs to evolve from the aviation tradition. It needs a fatality accident (loss) probability at least as low as one in a hundred thousand. Commercial jetliners today are about 1 in 10 million.
My guess at how such an RLV would operate gives safety of flight the highest design priority. Preferably no staging events, i.e., single-stage-to-orbit. Horizontal takeoff and landing with refused takeoff capability. No in-flight engine starts unless the plane can fly back to safe landing in case of failure. Safe abort from anywhere in the trajectory with an engine out. A small number of engines. The safety optimum is 2 to 4 as for jet aircraft. Finally, redundant flight controls. Most of this comes straight out of federal air regulations for commercial jetliners. The technical challenges to achieve these attributes are daunting, and may take time.
How does NASA’s space launch initiative (SLI) fit into this picture? It is supposed to be paving the way for low cost and safe space transportation.
NASA is anxious to show its RLV requirements “converge” with those of the commercial world. If true, NASA believes commercial investors would put up a large share of the money for RLV development.
I believe commercial motivation to replace the new generation of satellite launchers just now coming into service is nil. Atlas III, Sea Launch and Delta III have had a couple of launches. Delta IV and Atlas V are yet to fly. These systems are mostly privately financed. Their investment has not been paid out. Replacing them with a lower-priced RLV is bad business. If prices are reduced, revenue is reduced.
NASA needs a shuttle backup or replacement, since the International Space Station (ISS) program is highly dependent on shuttle. If requirements are not convergent, NASA should pay to develop the shuttle backup or replacement. Options include: (1) Shuttle upgrades and safety improvements; or (2) A crew and cargo carrier launched by existing ELVs; it would carry half a dozen crew and a few tons of cargo. (Heavy cargo such as an ISS module could be launched on an ELV); or (3) A new RLV designed to do the shuttle job.
If the third option included human missions such as return to the Moon or exploration of Mars, the RLV business case for NASA would close. If ISS is the only mission, option 1 or 2 seems preferred. From NSS’s point of view, we want enough commitment to human missions to the Moon and Mars to adopt option 3.
A tourist RLV has little in common with NASA requirements. Convergence of requirements is a bad premise for SLI. If it’s true it would be a pleasant finding, of course. But it’s probably not true. Using it as a starting assumption does not help validity of results.
On the commercial side, investors need to know if there’s a market with enough demand to close a privately financed RLV business case. If suborbital vehicles that fly to space (>100 km) are a business success, commercial RLVs can evolve from there. To help stimulate early commercial ventures, one or more “mail contract” analogs could be adopted: NASA could buy instrumented flights of a suborbital vehicle as a R&D testbed. Or NASA could buy flights for human-tended microgravity missions, sort of a “super vomit comet” (KC-135). Only a few flights need to be bought: to reduce risk, provide a modicum of cash flow, and enhance investor confidence. These are small investments compared to overall SLI plans and could be readily added.
Also, commercial venturers need NASA’s technology help, especially for orbital vehicles. The main area is propulsion; others include thermal protection and flight environment prediction. Most NASA technology activities now planned are needed, and the technology part of SLI would continue as planned.
When? I have guessed that the first tourism RLVs are considerably smaller than NASA’s needs. I believe an orbit-capable one could be flying in about 12 years. If commercial aviation is a valid analog (it may not be, of course) a second or third model tourism vehicle would be flying by 20 years, and might have as much payload capability as NASA needs.
If NASA focuses on shuttle replacement or backup instead of trying to cudgel the aerospace industry into putting up the investment for its RLV development, a crew/cargo carrier for an existing RLV could be built in 4 years. If a new RLV is selected, six to eight years might be required. But development could start in a couple of years rather than waiting until 2005.
No Closed-Loop Life Support System
The third issue facing development of a spacefaring civilization is life support. Permanent outposts or settlements can’t afford to import life support supplies or equipment over the long term.
The current technology is adequate for the space station. It provides partial recycling of water and oxygen, using “physico-chemical” technology. It uses chemical absorbers and reactors, and physical processes such as distillation and reverse osmosis, to recycle water and scrub CO2 from air. Oxygen is reclaimed by water electrolysis and CO2 reduction. Hydrogen and carbon from these processes are waste products, not recycled.
There is no food production, and no recycling of wastes or garbage; these are returned to Earth.
For the International Space Station, the crew and operations resupply requirement is about 10 kg per person per day. The ISS will typically have a crew of four; in 90 days it needs 900 kg per person; 3600 kg for the crew. This is easily within shuttle capabilities, even the capabilities of a crew and cargo vehicle flying on an ELV. There is little motivation to do better.
A Mars proto-settlement of 1,000 people is a lot different. Such a settlement is not feasible with this state of technology. Consider 1000 people, 365 days, at about 10 kg/day. This figures to 3.65 million kg (about 8 million lb) per year. Even at reduced launch cost of $1,000/lb, the delivery cost to Mars is at least $5,000/lb. The annual cost therefore is $40 billion just for life support. No government or consortium of governments will put up with such high cost, and it is out of the question for the private sector.
Bioregenerative technology is needed. This technology is also highly applicable to cleaning up our environment here on Earth.
A permanent outpost needs a closed micro-ecology or something close to it. This means full recycling of all life support supplies, including waste and garbage. Periods of “no opportunity” for Mars resupply last almost two years; transit times are six months or more. Not only is the cost infeasible for ISS-level technology, the masses to be transported are outrageous.
In a bioregenerative system, water and oxygen are recycled by semi-natural means, such as composting or oxidation of organic wastes, and condensation of water. CO2 is taken up by plants, and oxygen generated by photosynthesis. Plants produce food, and some may be ornamental or needed to make the micro-ecology stable.
Food production is by “farming” — hydroponics. Animal protein production is feasible in larger outposts. Wastes are completely recycled. Nothing is thrown away.
The life support and food production system must have long-term ecological stability. Such a closed-cycle technology is very poorly understood; Biosphere II showed how little we really know. Unfortunately, NASA is investing almost zero in this. A few years ago, NASA invested modestly. However, in today’s political climate, these investments are seen as applicable only to non-approved programs and are strongly discouraged.
It is likely that developing bioregenerative life support technology to a point of confident use, i.e., where space settlers could depend on it, will take longer to solve than the high cost of space transportation, perhaps much longer.
The research is not expensive; much of it can be done in a university setting. It needs to be pursued vigorously. This is a simple issue, closely related to NSS’s “1% for the Future” campaign. Congressional acceptance of the idea of “1% for the Future” would open the door to resumption of this very important research area.