by G. Harry Stine
Copyright 1981 by G. Harry Stine
Reproduced with permission of the G. Harry Stine estate
Chapter 7: Electric Rockets — Opening the Solar System
Some of the consequences of space activities as a result of the development of the build-up of both space facilities and the space transportation system were discussed in Chapter Five.
It’s a natural and almost trivial forecast to anticipate that the ready availability of space power would result in an increased demand for space transportation.
But it was not so obvious that the development of cheap space transportation from Earth to orbit would bring in its wake the relocation of earthbound industries and hence 60% of the United States baseload electrical requirements into space.
And few forecasters and planners have yet discovered that the availability of cheap and abundant electricity from the SPS system creates a climate for the development, phase-in and operation of totally new forms of space transportation other than the chemical rocket.
To some extent, this is presaged by the deep space freighter, a module consisting of an electric rocket engine that would be powered by the solar electric output of the SPS photovoltaic array that it propels from the LEO Base construction site up to the GEO Base assembly site.
Electric rocket engines have been around for several decades but have generally taken a back seat in the public mind. The requirement for rocket power has been for large, high-thrust rocket engines capable of performing what has been and will continue to be the most difficult and energy-consuming portion of space flight: the boost from the Earth’s surface to low Earth orbit where the space vehicle must fight its way against the persistent and strong pull of Earth’s gravity. The high-thrust characteristics of this type of rocket propulsion plus the absence of any well-developed technical means for transmitting energy to the rocket vehicle from any sort of a large and permanent energy conversation facility have made it possible to use only one type of rocket propulsion system: the chemical rocket.
Everything we’ve launched into space to date has been flown under the thrust of a chemical rocket. Usually, the oxidizer has been liquid oxygen (LOX) although the Titan-n and Titan-Hi launch vehicles used nitrogen tetroxide. Solid propellant rocket motors have been used in the NASA Scout, as the third stages of all the U.S. Delta launch vehicles, and as the boosters for the Titan-HIC and the NASA space shuttle. The solid propellants of this type of rocket normally consist of potassium perchorate as the oxidizer and some sort of metallized synthetic rubber formulation as a fuel, both propellants being mixed together in solid form. Liquid propellant chemical rockets have used numerous fluids as fuels. The most common of these has been RP-4 which is a form of highly refined kerosene. Other liquid fuels have included ethyl alcohol, unsymmetrical dimethylhydrazine, and the most powerful of all liquid fuels, liquid hydrogen (LH).
There have been two basic problems in the use of chemical rockets of all types using both solid and liquid propellants.
First of all, there is only so much energy that can be obtained from the combustion of a fuel and an oxidizer, and this energy shows up as exhaust velocity (which should be as high as possible) and in the rocket performance factor called “specific impulse” (thrust produced per unit weight of propellant consumed per second). The propellant combination with the best energy efficiency—liquid hydrogen and liquid oxygen—is already in use, and the only way to increase its exhaust velocity and specific impulse is to burn the propellant combination in a rocket engine at the highest possible temperature and pressure. The most energy efficient hydrogen-oxygen rocket engines used to date are the main engines of the NASA space shuttle orbiter. These Space Shuttle Main Engines (SSME’s) operate with a combusion pressure of 3000 pounds per square inch. This pressure is equivalent to that used for the storage of welding gases in those heavy steel tanks that accompany every gas welding outfit. The high combustion pressure—the highest that’s ever been used in a large rocket engine system—creates difficult engineering problems in the design of pumps and turbines which have to deliver these exotic super-cold propellants to the rocket engine at these pressures. With what rocket engineers and propellant chemists know today, the SSME’s represent the absolute ultimate in liquid propellant chemical rocket engines. At pressures and temperatures only slightly higher than those in the SSME’s, dissociation of the combustion product—water—takes place, thus robbing the system of any additional energy that’s gained by increasing combustion pressures and temperatures. We’ve just about reached the end of the road insofar as being able to increase the exhaust velocity and specific impulse of chemical rocket engines.
Secondly, every space vehicle propelled by chemical rocket engines must carry along with it all the propellants required to complete the vehicle’s flight. For Earth-to-orbit space vehicles, this means that more than 90% of the vehicle’s launch weight must be made up of rocket propellants. Deep space vehicles can operate with less of a percentage of their mass being required by rocket propellants. However, regardless of whether the space vehicle is a shuttle or a deep spacer, the common and primary part of a chemical rocket’s design and structure is its large, bulky, and heavy propellant storage tanks.
The same two drawbacks also affect the nuclear rocket engine. Because of the nuclear test ban treaty of August 5, 1963 that prohibits nuclear tests or explosions in the Earth’s atmosphere, under water, and in space, the extensive development carried out on the nuclear rocket engine by the United States Atomic Energy Commission and NASA slowly wound down and come to a halt in the early 1970’s. Billions of dollars of research and development had been poured into the nuclear rocket engine project for more than twenty years and had resulted in a series of successful nuclear rocket engines beginning with the experimental Kiwi-I and culminating with a nuclear rocket engine designed for flight, the Nerva, which would have delivered 250,000 pounds of thrust with a specific impulse 2.5 times that of the SSME’s.
Basically, the method of operation of a nuclear fission rocket engine is simple. A nuclear reactor is used to heat hydrogen—carried as liquid hydrogen aboard the space vehicle. The hot hydrogen at more than 4500-degrees Fahrenheit is then expelled through a rocket nozzle to produce thrust.
The nuclear rocket engine, had its development been permitted to continue, would have made possible extensive space operations above the Earth’s atmosphere. It’s still in contention for possible future use in space where there is no possiblity that its exhaust could contaminate the Earth’s atmosphere. However, even though a nuclear rocket engine need carry along only one propellant—liquid hydrogen as “working mass”—it still must carry along its own energy source. And secondly, its specific impulse is limited to only about four times that of chemical rocket engines.
Because of its extremely high exhaust velocity, the electric rocket engine has a very high specific impulse in terms of thrust produced per unit weight of propellant consumed per second. The electric rocket principle is the same, regardless of the category of the electric rocket engine—arc, plasma, or ion. Electricity is used to produce charged particles such as electrons or ions which are then accelerated to very high velocities by electromagnetic or electrostatic fields and ejected from the rocket engine to produce thrust. But electric rockets don’t generate very much thrust and must operate in a vacuum. To date, electric rocket engines have been used on satellites and manned space craft as attitude control thrusters because high thrust is not required for this application. Electric rockets will be used both in the Deep Space Freighters of the SPS program and on the SPS units themselves in order to provide attitude control of the big photovoltaic arrays. Here again, high thrust is neither desired nor required, but high efficiency is. And, unlike chemical rocket engines, the electric rocket engines that will be used in the SPS program do not require that all their energy supplies be carried along in the form of propellants; they can get their energy directly from the Sun itself via the solar arrays of the SPS.
This makes the electric rocket very efficient for deep space use once the SPS system is in place.
Deep space flight from orbit to orbit in the Earth-Moon system doesn’t require high thrust rocket engines; it just requires steady, persistent, highly efficient thrust such as that produced by the electric rocket engine. In fact, deep space flight with constant electric rocket thrust, even at a fraction of one-gee acceleration, can result in trip times that are significantly shorter in duration than the usually-considered sort of rocket flight—a short period of high thrust followed by a long period of coasting flight terminated by a final short duration flight phase of high thrust to match velocities with the destination.
Electric rockets have been confined to such prosaic uses as attitude control to date because there’s been a major technical problem of providing the electric rocket with a low-mass, high-efficiency electric energy source in space.
The SPS provides such an energy source.
The SPS also means that the deep space vehicle doesn’t have to carry along the electric energy source, only a small amount of reactive mass such as argon or whatever the electric rocket engine uses to produce its charged particles that are accelerated to produce thrust.
Furthermore, a lot of electric energy can be provided, permitting the use of much larger electric rocket engines than formerly possible.
We have visualized the solar power satellite thus far only as an energy source for Earth. But the same technology and the same design could also be used and will be used as a space power source. We’ve alluded to the forecast that industries would begin moving into space in the early 21st century because of the abundant and low-cost energy available in space from an SPS system. This means that some SPS units won’t be directing their power beams toward rectennas on Earth but toward rectennas in space.
And instead of a single SPS projecting a single power beam, it will be projecting several from a multitude of transmitting antennas to a large number of smaller space rectennas mounted on space facilities . . . and on space vehicles propelled by electric rockets.
Naturally, it isn’t absolutely necessary that a large, centralized system such as an SPS be used. Solar electric energy could be obtained by space facilities and space vehicles from large photovoltaic collectors that are integral with their design. It’s just that the large SPS unit is more efficient and will provide power at a lower cost to space facilities and vehicles, at least in the Earth-Moon system during the early years of the 21st century.
Now it becomes possible for deep space vehicles to venture as far as lunar orbit carrying only one working propellant—and not very much of that—for its electric rocket motors. The system could be built today, but it can be operated only in space after the SPS system is in part a reality. The SPS unit would have a small, steerable transmitting antenna. The space vehicle would carry its own rectenna— and it can be much smaller and use a higher density power beam that is possible for Earth use—as well as a pilot beam that would tell the SPS where the ship is and where and how to direct the individual beam that’s providing electric power for the ship.
It also means several SPS units—probably originally fabricated from terrestrial materials but later built from extraterrestrial materials—that are dedicated to providing electric energy exclusively to space facilities and vehicles.
This ready availability of cheap solar electric energy also makes possible the use of several other types of propulsion systems. This includes one that doesn’t use any propellant mass whatsoever.
The first suggestion of using a catapult device to propel a vehicle in space probably was discussed by none other than the French author, Jules Verne, in his book, De la Terra a la Lune, (From the Earth to the Moon), first published in 1870. Although Verne’s book wasn’t the first to talk about travel in space, it was the first to be written using the known science and technology of the day as its background. Verne’s catapult was a huge cannon, the Columbiad. The space vehicle that was launched by the Columbiad carried no propulsion systems; all the energy for the circumlunar flight was imparted by the cannon’s propellant charge, a fulminate of nitrocellulose Verne called “pyroxyle.” The Columbiad was a space catapult.
Robert A. Heinlein, in his 1947 Saturday Evening Post short story, “Space Jockey,” and in his 1949 novella “The Man Who Sold the Moon,” suggested the use of an electric-powered Earth-surface catapult running up the east face of Pikes Peak in Colorado as a means of eliminating the need for a two-stage Earth-to-orbit shuttle in favor of a catapult-launched SSTO shuttle. In his 1966 novel, “The Moon Is a Harsh Mistress,” Heinlein postulates the use and consequences of a solar-electric lunar surface cargo catapult.
However, space catapults didn’t begin to receive intense attention until Dr. Gerald K. O’Neill became involved in his work on space colonization at Princeton University in the early 1970’s. Dr. O’Neill publicized the concept of the “mass driver,” a solar-electric space catapult that could be used both on the lunar surface and in deep space. Since 1976, Dr. O’Neill and his associates at Princeton have carried out additional research work on the “mass driver” which is based upon the established technology of the linear electric motor or sequential solenoid, both of which have been widely used in industry for decades. Today, space advocates use the term “mass driver,” most of them not knowing that this is an old concept.
Perhaps the biggest difference between the historic space catapults and Dr. O’Neill’s mass driver is the size and frequency of the pay loads launched. Classic space catapults have considered launching multi-ton space vehicles or cargo payloads much like the catapults used to launch aircraft from warships. Dr. O’Neill’s mass driver would launch payloads of about 2 kilograms every few seconds in a constant stream toward the destination.
The biggest problem with space catapults and mass drivers is not the energy required to make them work, that energy will be readily available from an SPS system. It’s the question of manipulating the catapult’s payload when it reaches its destination, especially if said payload has no on-board propulsion system. An Earth-to-orbit catapult launching an SSTO shuttle doesn’t have that problem because the SSTO shuttle has propulsive energy to match orbits with its LEO destination. A lunar surface catapult designed to lob unmanned payloads to Earth doesn’t have that problem because the payload or its container can be designed to be slowed down by atmospheric entry and land in the ocean. But everywhere a space catapult or mass driver might be considered for use in the early years of its deployment, there is a problem of catching the payload.
An unmanned, unpowered payload of any size launched from orbit to orbit or from lunar surface to orbit simply returns to the launch site unless an additional change of energy is imparted to it at the proper time and place in its trajectory. It’s like a cannon shell. It’s like the manned projectile launched from Jules Verne’s Columbiad.
Technically, the problem of catching the payload or of imparting an energy change to it can be solved. Dr. O’Neill has designed a catcher for his two-kilogram mass driver payloads. With abundant energy available from an SPS system, there are several approaches that can be taken toward imparting an energy change to an unmanned, unpowered payload to permit it to arrive at its space destination with zero velocity difference between it and the destination.
One of the most interesting potential solutions comes from something that was the brainchild of Dr. Arthur Kantrowitz, formerly with AVCO and now at the Thayer School of Engineering at Dartmouth College. It’s also an interesting potential propulsion system for use anywhere in the Inner Solar System where the energy of the Earth-orbit SPS system can be used.
Dr. Kantrowitz proposed the concept of the laser-energized rocket in the late 1970s when the potential of the high-powered lasers being developed for possible military uses was under discussion by many people.
The laser rocket would use a tightly-focused beam of energy from a high-energy laser situated as a fixed facility on a planetary body or in space. The laser beam would be directed at the rear end of a space vehicle where the energy of the beam would vaporize a solid material to provide a gaseous reaction mass to be expelled in rocket fashion from the vehicle. This is similar in concept to the SPS-energized electric rocket except for the fact that the laser rocket would be simpler and would eliminate many of the energy conversion steps of the SPS-electric rocket.
Because of the ability of a laser to generate an energy beam that can be tightly focused, thus preventing the dissipation of the beam over long distances and the increased possibility of reception of a majority of the beam’s energy at extreme distances, the laser rocket offers great promise as a propulsion system for use in the initial phases of exploitation of the planetoid belt for extraterrestrial materials. The energy for the power laser in Earth orbit would come, of course, from an SPS unit. The laser could provide energy for a space vehicle all the way out to the planetoid belt and perhaps to the planet Jupiter. In fact, such a laser method of squirting energy over long distances could be used to provide the necessary energy from established facilities in Earth orbit for manned facilities and vehicles throughout the Inner Solar System during the first decades of the 21st century.
Laser power beaming may, in the final analysis, be the optimum method for beaming SPS power to the Earth’s surface.
Again, we discover that we’re dealing with a “boot strap system”—it becomes possible to build systems upon systems to create synergistic multiplication of capabilities far beyond that which we could hope to achieve with one system alone.
With the energy available from the expanded SPS system in geosynchronous Earth orbit, it now becomes possible for us to provide energy for our use anywhere in the Inner Solar System. This will make it much easier to travel from point to point in space, and it will make it much, much easier to maintain populated facilities wherever we wish to put them.
We have just extended our concept of energy and its relationship to social organization from its original earth-bound application. We’ve extended it into the Solar System. Where we have energy, people can live and work. Where we have energy, we can travel. Where we have energy, we can alter the natural order to make it more useful to us as human beings.
With the propulsion devices made possible by the SPS system in Earth orbit and the consequence that we will be able to travel to and work in any part of the Inner Solar System where it’s possible to use solar energy, before the 21st century is half over we will have reached the point where we no longer have to depend upon SPS units in GEO for energy beamed to the Earth.
We will be able to construct extremely large Solar Power Satellites at the terrestrial-solar libration points where they will be able to beam power to the Earth-Moon system.
A libration point is a “special solution” to the old “Three Body Problem” of celestial mechanics. Sir Isaac Newton in his classic 1687 statement of the laws of motion and gravitation, Principia, showed us the solution to the problem of the gravitational interaction of two bodies. But there is no known general mathematical solution to the behavior of three celestial bodies of sizes such that their individual gravitational fields affect the other bodies in the three-body system. There are, however, five “special solutions” to the three-body problem.
Figure 1 shows the Earth-Moon system drawn to scale. The location of geosynchronous orbit is shown so that we can get some perspective on distances—but not travel energies and times required. There are five points in the Earth-Moon system where another object ranging from a small satellite up to a Moon-sized celestial body could be theoretically located and retain its position with respect to both the Earth and the Moon. In these five locations, the gravity fields of all three bodies would be in balance.
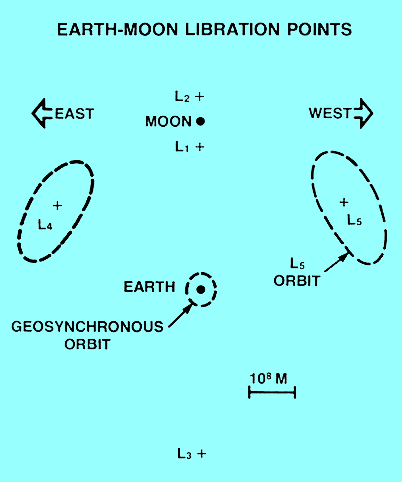
Figure 1
These five locations are known as “libration points.” Actually, they are regions rather than precise locations. They are also known as “Lagrangian points” in honor of Joseph Louis Lagrange (1736-1813), the French geometer and astronomer who first suggested this special solution to the three-body problem.
The First Lagrangian Point, shown as L-1 on the drawing, is located on a line between the Earth and the Moon and approximately 76,000 kilometers from the Moon. The Second Lagrangian Point, L-2, is also located on a line from the Earth to the Moon but is about 71,000 kilometers outside the orbit of the Moon. The Third Lagrangian Point, L-3, is on the Earth-Moon line but located in lunar orbit 180-degrees away from the Moon on the other side of the Earth.
The first three Lagrangian Points are “unstable”—any object placed at these Lagrangian Points will eventually wander away from them because of fact that the orbit of the Moon isn’t exactly circular and because of the gravitational pull of the Sun.
But this isn’t the case with the Fourth and Fifth Lagrangian Points, L-4 and L-5. The Fourth Lagrangian Point is located in the lunar orbit 60-degrees ahead of the Moon, while the Fifth Lagrangian Point is also in lunar orbit but 60-degrees behind the Moon. L-4 and L-5 are stable libration points and are also known as the “Trojan Points.” We know that L-4 and L-5 are stable because the discovery of the Trojan planetoids (named after the heroes of Homer’s Illiad) at the L-4 and L-5 points in Jupiter’s orbit about the Sun. The Trojan planetoids have obviously been there for a long time. Thus, the Trojan Points are super-stable locations, but they are not the only stable orbital locations even in the Earth-Moon system.
Technically, it would be possible to put any number of objects in lunar orbit or any other orbit and have them stay in place for a long period of time—hundreds of years or more in some cases—with only minor orbital adjustments with electric thrusters. Any number of satellites can occupy a given orbit; the rings of Jupiter, Saturn, and Uranus are proof of this. (Earth has no rings; Dr. Clyde W. Tombaugh proved this as part of his extensive search for small natural satellites of the Earth in 1953-1959.)
As implied, Lagrangian libration points exist for every major body in the Solar System and for every planet-satellite orbit as well. There are equivalent Lagrangian Points in the Sun-Earth system, the most stable (because of the tremendous mass and strong gravity field of the Sun) being the Sun-Earth Trojan Points. There are mercurian Trojan Points, venerian Trojan Points, aerean Trojan Points, etc.
The Trojan Points in the Inner Solar System are going to be occupied in the first half of the 21st century. Among the many things that will be located at the various Trojan Points are Solar Power Satellites of very large size, fabricated from extraterrestrial materials, and sending their energy to various locations on radio and laser beams.
The Trojan Points in the Sun-Earth system are attractive as locations for such large SPS units because the relationship between the Sun and the SPS and between the Earth and the SPS remain reasonably constant. This means that a Trojan Point SPS doesn’t need to be continually re-oriented to keep its photovoltaic panels pointed toward the sun or its energy transmitter aimed toward its consumer’s rectenna.
The Trojan Point SPS system of the 21st century is only a dream today. But by 2050 or 2060, such a system could be supplying the Earth-Moon system with whatever power it needed from SPS units in the Earth-Moon Trojan Points. Additional energy would be coming from SPS units in the two Sun-Earth Trojan Point locations. The Trojan Point SPS system would also include units whose job is to provide in-flight energy to deep space vehicles via power beams, and these transportation-dedicated SPS units would have several transmitting antennas or use a single antenna with special phasing circuitry.
(Antenna design borders on black magic although it is a very solid technology based firmly on mathematical foundations. It’s possible to get antennas to do a number of magic tricks depending upon their design. For example, one antenna can transmit to several receiving units, each receiver being illuminated by a separate antenna beam. And, since a good transmitting antenna is also a good receiving antenna, a single antenna can be used for both purposes by multiplexing or rapid switching.)
All that is necessary is to be willing to accept the risks, expend the capital resources, organize the effort, and get started in the 1980 decade on the development work necessary to prove out the essential technologies of the first SPS system in geosynchronous orbit. Once we have started that task in order to solve the energy crunch, the consequences are so attractive and the potentials are so enormous that the entire Inner Solar System is opened up to utilization by the human race.