Colonies in Space:
Chapter 16 – Colonizing the Stars Chapter 16 – Colonizing the Stars
Colonies in Space
by T. A. Heppenheimer
Copyright 1977, 2007 by T. A. Heppenheimer, reproduced with permission
Chapter 16 – Colonizing the Stars
In contemplating flight to the stars, the best understood fact is also the most important: the stars are far away. The closest are a million times farther than the closest planets, which in turn are about a million times farther than the distances that might be reasonably covered with a ten-speed bike. The comparison of a planetary mission to a ten-speed is not necessarily the measure of the technical advances needed to build suitable starships. There are no atomic bicycles but there have been plenty of proposals for atomic rockets and in designing a starship, the principal problem is to find a suitable means to apply nuclear energy to rocket propulsion.
To carry out interstellar missions in a reasonable time, such as a few decades, we need rockets with 1000 times the exhaust velocity of our current chemical ones, at least a few tens of millions of feet per second. The energy in the propellant has to be a million times greater than in today’s chemical propellants and nuclear fuels have the happy property of possessing a million times more energy than chemical ones, which is why a one-megaton nuclear bomb weighs only one ton. But the problem of applying this energy to rocket propulsion has bedeviled more than one propulsion engineer.
There were proposals for nuclear particle emitters, the advocates of which believed they could produce 30 million feet per second of exhaust velocity. There were fission reaction particle emitters shaped into huge hemispheres, as well as radio-isotope sails of large area and thin, light construction. The propulsion specialist Robert Corliss has described their state-of-the-art as “none” and their usefulness as “not a feasible propulsion system.”
In 1960 Robert Bussard proposed the interstellar ramjet. This idea involved a starship which would scoop up the incredibly thin, diffuse hydrogen of interstellar space and heat it and compress it to the conditions found in the center of the sun. It would undergo thermonuclear fusion, yielding helium, which would exhaust out the back. Bussard’s concept had the advantage of being ingenious. It had the disadvantage of being wrong.
He assumed a drag-free starship, which in his context is a little like assuming a drag-free airplane. If such a plane could be built, it could fly at high speed just by burning atmospheric smog in its own ramjet. Such a smog-burner, of course, would give very weak thrust (smog is never that bad) and so would Bussard’s ramjet. Hydrogen, at the conditions found in the solar interior, yields energy at the rate of two ergs per second per gram. A cooling bathtub releases energy a thousand times more rapidly. In terms of power-to-weight ratio, a Roman galley full of slaves would be more efficient. Even when the slaves were asleep you could get more energy from their body heat.
The topic of interstellar flight passed into fiction, such as “Star Trek.” Too many engineering proposals had invoked too much hypothetical physics, sometimes together in anachronistic combination with current technology. It was as if Jules Verne had written of a steam dirigible carrying antigravity material for a lunar flight.
In 1971 Krafft Ehricke tried to predict the most advanced mission which might be undertaken with the technology then known. He chose the gas-core nuclear rocket for propulsion, a concept under study, which would contain a plasma of uranium inside a transparent wall, cooling the wall with the aid of a space radiator and achieving exhaust velocities up to 200,000 feet per second. He foresaw a multistage gas-core rocket, launched with the aid of gravitational tricks involving both Jupiter and the sun—and after 40 years reaching the distance of 0.1 light-year, one-fortieth of the way to Alpha Centauri.
At about the same time, Keith Boyer and J. D. Balcomb, leading nuclear propulsion specialists at Los Alamos Scientific Laboratory, offered the following assessment:
Specific energies of nuclear reactions are so high that system performance is limited by design constraints well before the specific-energy limit is reached. For example, the specific energy of uranium in fission [corresponds] to a maximum exhaust velocity of 40,800,000 feet per second, whereas the specific energy of deuterium plus tritium in fusion [corresponds ] to a maximum exhaust velocity of 83,600,000 feet per second. Such exhaust velocities are only of theoretical interest. Many factors restrict any practical consideration to values up to 300,000 feet per second. [From American Institute of Aeronautics and Astronautics paper 71-636.]
A few years earlier in 1965, James Strong had said much the same thing in his book Flight to the Stars:
The successful orbital flights of astronauts have tempted space travel enthusiasts to compare them with the pioneering days of heavier-than-air flight at the beginning of the century. The analogy is misleading if it leads others to conclude that, sixty years from now, spaceliners will be crisscrossing the Solar System [as if they were] jet aircraft today. Men’s first ventures into space would be better described as comparable to the hot-air balloon ascents in the eighteenth century. In effect, [some] 180 years must elapse [before] the luxury of fast interplanetary travel. Yet even by A.D. 2140, it would be surprising if a propulsion system for star flight were to exist. Another seventy years should see the broad outlines of a power unit emerge and take shape.
However, it did not take seventy years, but more like seven, for “the broad outlines to emerge and take shape”—not the twenty-third century but the year 1972. That year certain research findings were declassified, and for the first time it became possible to discuss star flight in terms of real feasible methods. At the same time it was found that there was intensive work under way, aimed at building what could only be described as laboratory experiments on the main thrust system for a starship.
This work is in controlled fusion. Since the invention of the laser early in the 1960s, it had been known that it was possible to take a pellet of liquefied thermonuclear fuel—deuterium and tritium, the heavy isotopes of hydrogen—hit it with a laser beam, and raise it to the temperature required for ignition, about 100 million degrees. This laser-zapping would not give a practical fusion reaction through merely heating the fuel, but it would do so if the laser were used to compress or implode the fuel to 10,000 times its normal liquid density. Although by the late 1960s laser fusion was being widely discussed, the most important concepts, including implosion, were made known only when the 1972 action of the Atomic Energy Commission declassified this line of research.
How can lasers compress a liquid? To do this while initiating fusion, it is necessary to deliver a pulse of laser energy to the pellet totaling some 10,000 joules—the energy of a small car at ten miles per hour. The energy must be delivered in the form of multiple beams focused to cover the entire surface of the pellet, which is less than a millimeter in diameter, and the delivery must take place in less than a nanosecond, a billionth of a second. This blows off the outer layers of the pellet at extremely high speeds. As the blown-off material accelerates, it generates a reaction force (by Newton’s Third Law) that compresses the interior of the pellet. The system acts as a laser-driven spheroidal rocket whose payload is the rapidly contracting pellet interior. The imploding matter is accelerated inward to a velocity 50 times higher than Earth escape velocity. It collapses inward until internal pressure from its electrons brings the implosion to a halt. By then the pressure has been raised to a trillion atmospheres and the temperature and density are such that fusion is initiated.
Not all the mass of the pellet undergoes fusion. The fraction which does (and therefore the energy released) depends on the energy of the laser. Fortunately the fusion energy released increases more rapidly than the required ignition energy, so if the laser is powerful enough, the fusion process becomes efficient. To demonstrate laser fusion in the laboratory, there is under way currently a program of building a laser capable of delivering 10,000 joules to a pellet in 100 to 500 picoseconds (trillionths of a second) or with modifications, 50,000 joules. This facility at California’s Lawrence Livermore Laboratory is to go into operation in 1977.
The most difficult problems involve the development of these large powerful lasers. For application to a starship or to the commercial generation of electricity, it will be necessary to have lasers with at least several hundred thousand joules in the pulse. For laboratory experiments, the big lasers are made from a well-understood material, neodymium glass. The problem is that all lasers are inefficient, requiring many times more energy to excite them than is subsequently delivered in the laser beam. For neodymium glass, the efficiency is about 0.1 percent. What is wanted is a gas laser with efficiency of at least 5 percent and work is now under way to find the best solution. Carbon-dioxide lasers appear to be particularly promising, offering efficiencies up to 10 percent, and are receiving nearly as much attention as neodymium glass. Other laser media which appear promising include hydrogen fluoride, argon, krypton, xenon, iodine, oxygen, and the vapors of the metals mercury, copper, or manganese.
It is too early to tell which of these media will be best but to aid in making the choice, Los Alamos scientists are working on a 100,000-joule gas laser facility which will be able to test these various media. To excite the lasers, to bring them to the condition to produce their pulses, considerable attention is being paid to the use of intense beams of high-energy electrons. These can excite large volumes of gas in a few nanoseconds. The electron beams themselves may serve to produce implosion and fusion of the pellets—a possibility which is attractive since electron-beam generators are more efficient than lasers.
As a result of all this, it now is possible to describe the broad outlines of a starship engine.
A useful starting point is the propellant tank, which contains a mixture of liquid deuterium and tritium. Fuel is pumped to a pellet-preparation system, where it is formed into a large number of small hollow spheres, resembling bubbles, each the size of a grain of sand. The pellets are frozen solid to aid in their handling and passed on to the injector.
The injector is a rapidly rotating device to accelerate pellets to about 10,000 feet per second and eject them into the engine thrust chamber. The high velocity allows a high frequency of fusion microexplosions, increasing the thrust of the engine. To do this, the injector is a small version of the rotary pellet launcher. It injects pellets at a rate of 500 per second.
Each pellet passes down a tube 300 feet long on its way to the thrust chamber. This allows its trajectory to be examined and corrected. The tube is equipped with optical sensors and lasers, which can be focused on one side or the other of the pellet. This blows off small quantities of its mass, producing a thrust which changes the pellet’s direction of flight. In this manner the pellet is guided to the proper direction. At the same time its arrival time is predicted, so the laser can be primed to fire during the interval of 10 nanoseconds when the pellet will be passing the fusion point.
The thrust chamber consists of front and rear superconducting magnetic coils, mirrors, a pick-up coil, radiators, and structural tie bars. The superconducting coils produce strong magnetic fields to redirect the charged particles produced in the microexplosion, forcing them to stream out the back to produce thrust. These coils and other structural components are exposed to intense floods of X rays and neutrons. They can be protected from damage by having their exposed surfaces shielded with layers of beryllium and beryllium oxide. The coils and tie bars also must be cooled and for this there are layers of heat pipes inside the shields. These transport heat to the space radiators, where it is rejected at 2300°F. Insulation and recirculation of liquid helium then are enough to maintain the superconductivity of the coils.
The pellet enters the chamber and the laser fires. Its pulse is split into eight subpulses, which are directed onto eight mirrors mounted on the tie bars. The mirrors also are cooled with radiators. The subpulses converge on the pellet as it passes the fusion point, and about 25 percent of its mass undergoes fusion. About one-third of the energy released is in X rays and fast neutrons, the rest being in charged particles. These form a fusion fireball, a mass of intensely energetic plasma, which expands outward. The plasma soon encounters the magnetic field produced by the front and rear coils and pushes it outward, as if that field were a balloon into which air was being blown.
As it expands outward, the magnetic field is pushed past the pickup coil, which generates an induced voltage. The energy transferred to the pickup coil is about 5 percent of that generated in the microexplosion and it appears as electric energy. It is extracted from the pickup coil, transported down a transmission line, and dumped into an array of capacitors forward of the laser. The capacitors store enough energy to excite eight firings of the laser, so if one or a few pellets fail to undergo fusion, there still will be energy to fire the laser.
The plasma from the explosion is prevented from reaching any part of the thrust chamber structure by the magnetic fields. Instead, it is directed out through the rear coil. The thrust is 15,000 pounds; exhaust velocity is 30,000,000 feet per second, or 3 percent of the speed of light—a speed which is written as 3 psol.
The laser is a gas-filled cylinder 400 feet long by 20 feet in diameter. Passing through the central volume of the cylinder are heat pipes, which extend on either side of the cylinder to form the main starship radiator in the shape of two “wings.” These run the length of the laser and operate at 2300°F, to maintain the laser at this temperature. Surrounding the laser are the elements of the electron-beam generator and its power supply fed from the capacitor bank. There is a small nuclear reactor to provide start-up power and to operate other onboard systems.
This is the starship engine. It is founded on well-understood principles of physics and it is essentially as simple as any chemical rocket motor. Quite likely within 10 years the problem of the laser will be well in hand, so we will know what gas to put in it. Then it will be a matter of engineering design and development, similar to that associated with any new type of rocket. The result will be the highest-performing engine conceivable in terms of our understanding of physics. It will not be able to give speeds close to that of light, justifying the efforts of the platoons of mathematicians who have combined two trendy topics by studying the application of the theory of relativity to rocket flight. As it is, we will have to plod along at something like 10 psol—enough to get from Earth to the moon in 13 seconds. This means 43 years to the nearest star; a bit long, but manageable.
The longest planetary missions yet undertaken have involved sun-circling spacecraft such as Pioneer 6, launched in 1965 and still going strong. The longest ones seriously studied were a Jupiter-Saturn-Uranus-Neptune mission, in the Grand Tour program, with a duration of 13 years. The shortest interstellar missions could be only a few times longer than the longest planetary missions that are the stock-in-trade of JPL, the Jet Propulsion Laboratory. The initial interstellar missions, which will be unmanned, may be quite similar to the more advanced unmanned planetary missions which have been undertaken or may be flown in years to come.
Present-day missions will contribute to the stellar program particularly in the areas of computers, long-lived energy sources, and communications. For the initial missions, one key need will be an onboard computer which can be kept operational for several decades, while exhibiting the characteristics of artificial intelligence. Even today this problem appears well in hand. During its work on the Grand Tour program, JPL scientists were working on the STAR (self-testing and repair) computer, which would check itself out and replace failed units with spares when necessary. Such a capability itself requires a degree of artificial intelligence—the ability to recognize problems and exercise judgment on the basis of inputs from sensors.
JPL and the Stanford Research Institute are continuing to study artificial intelligence. For instance, on future Mars rovers it will be desirable to set down a tracked or wheeled vehicle which will take care of itself. If it comes to a crevasse it should not blindly fall in or, recognizing the danger, have to call up Earth: “Mission Control, there’s nothing underneath the terrain-sensor stuck out in front, what should I do?” Instead, it should be able to recognize a crevasse when its TV cameras see one and maneuver around it. Well before the first starship is to fly, all these problems should be solved. Computer designers can do wonderful things with integrated circuits and can store unprecedented amounts of data in magnetic-bubble memories.
Communications will of course be interstellar in scope and will rely heavily on the techniques described in Chapter 13. One of the best reasons for building Project Cyclops will be to use it for communication with starships, just as the planetary program stimulated the building of conventional radio telescopes. A 100-meter paraboloidal antenna on the starship, fed with a megawatt of power and beaming a narrow-band signal at a frequency within the “water hole,” will provide all the communicating power needed, and these systems have existed at least since 1960.
What instruments would such star probes carry? The large antenna would double as a radio telescope and would be a major instrument. The major item would be a large optical telescope carrying equipment to make observations in the ultraviolet and infrared, as well as at visible wavelengths. It would be equipped with the fullest range of instruments available to the modern astronomer: photometers, spectroscopes, radiometers, filter wheels for observation at selected wavelengths, polarimeters and image tubes, to name only a few. There would be arrangements to exchange lenses to permit a wide range of magnifications and fields of view. To permit even greater flexibility there would be a large mirror forward of the telescope to reflect the image of celestial bodies. Then the telescope itself need not swivel or turn—the mirror would be moved instead.
All this would be under the command of the starship computer. The computer would direct observations of the target star and other celestial objects of interest from the beginning of the flight. With months and years required for a signal from Earth to reach the ship, its instruments could do useful science only with a high degree of onboard autonomy. The computer would be primed to recognize anything new or interesting, to follow up the discoveries with more intensive observations using different instruments as appropriate, and to send back all the data over the radio link.
In the last five or ten years before arrival at its destination, the star probe’s attention will increasingly turn to observing its target star and the near vicinity. At a distance of about a light-year, the star will be resolved as a disk rather than as a point of light. At about the same distance under highest magnification, the near vicinity of the star could begin to show luminous points which would be identified as planets. With 0.1 light-year to go, or one year to arrival, the largest planets would also show themselves as disks and the smaller planets or largest satellites would be evident. With a month to arrival, planets the size of the earth would show disks. At 10 psol, the starship would spend a full twenty-four hours in the innermost billion miles of the star system with the mirror forward of the telescope busily scanning the planets and satellites previously discovered and the data storage system soaking up the findings which guide the computer in its decisions and which subsequently will be relayed time and again to Earth, so that there is no danger of data loss.
This brings up the interesting question of how the missions should be conducted. An immediate possibility is to equip the ship with detachable spacecraft—orbiters, landers, sample returners; to provide it with extra propellant so it can slow down and maneuver at the destination and to let it fly from planet to planet (if it finds any!), visiting a new one every month or so while dropping off these spacecraft as its calling cards. Such an approach would certainly return vast stores of information and would give us a picture of the nearby stars and their planets more intimate than the one we now have of our own solar system.
The problem is that such rendezvous-class missions would need either more fuel or more time than the alternative, the fast-flyby missions which would send the star probes barreling through full tilt. With a desired mission velocity of 10 psol and a rocket exhaust velocity of 3 psol, it will take twenty times the starship mass in propellant. If we wish to speed up to 10 psol and then slow to zero at the destination, it will take 400 times the starship mass or if the propellant mass is to be kept at 20 times that of the starship, the mission time will be doubled.
At JPL it is a policy when visiting a new planet for the first time that the mission will be a flyby. Perhaps that policy will extend as well to the first star probes; however, hopefully at least a few rendezvous-class missions will fly, even accepting the longer flight times, to some of the nearer or more interesting star systems.
With such excellent onboard equipment, even the fast flybys will return data characterizing the star systems better than we now know our own solar system from telescopic observations only. It will be possible to detect and track planets and their satellites, determining their diameters. By following their motions, their masses as well as the details of their orbits will be found. Their temperatures will be measured and mapped at the telescope. Spectroscopic measurements will give the densities and compositions of their atmospheres, including particularly the presence of water or oxygen.
In the hours of closest approach color photography will be possible, showing surface features as small as 30 miles across. Major storms or other atmospheric features will be visible in detail as will many features of the surface. Polar caps, craters, volcanoes, rift valleys, mountains will all be seen—as will seas, continents and islands, lakes and river valleys, if they are there. Even if a planet is shrouded in cloud, it will be possible to study the surface using radar with the onboard antenna. And the methods described in chapter 15, used for determining the surface composition of asteroids, will serve also for these new applications.
Such starships will face hazards caused by the space environment. At 10 psol each molecule of interstellar gas will be a cosmic ray and particles of interstellar dust, a micron or so across, will strike with the intensity of sand blown in a tornado. Telescope lenses will need to be protected with glass plates, which can be replaced automatically as they become scratched or pitted. Fortunately our present knowledge indicates that meteoroids most probably do not exist in interstellar space (will this assurance someday constitute famous last words?), but when passing through a star system, the spacecraft may encounter particles the size of a grain of sand. These will strike with the energy of a ton of TNT.
For protection from such impacts, the star probe will need a self-propelled shield vehicle to ride out ahead. It will probably be thirty feet across and ride six miles ahead, acting as an umbrella against the rain of dangerous particles. It will have to be heavily built to withstand the impacts and with onboard propulsion and navigation so it can return to its station after an impact. The shield vehicle will be one of the more critical items of the star probe, which leads to the question: what shields the shield vehicle? The answer will probably be that the probe carries several such items, which will give continuing protection if one is lost. The large standoff distance means these vehicles will not interfere with astronomical observations, and for some purposes they may even be helpful. By blocking the light from a star, they can make it much easier to look for its planets.
With starships at hand, what stars shall we go to? The litany of the planets is familiar: Mercury, Venus, Earth, Mars, and on to Pluto. The roster of the nearby stars is not so familiar. There are a total of fifty-nine within twenty light-years, which has often been taken as representing a limit for exploration. If our eighteenth-century ancestors had spent their time building starships instead of fighting the French and Indian War, we would now have the data from most of the missions. The first few are: Alpha Centauri, Barnard’s Star, Wolf 359, Luyten 726-8, Lalande 21185, Sirius, Ross 154, Ross 248, Epsilon Eridani, and Ross 128.
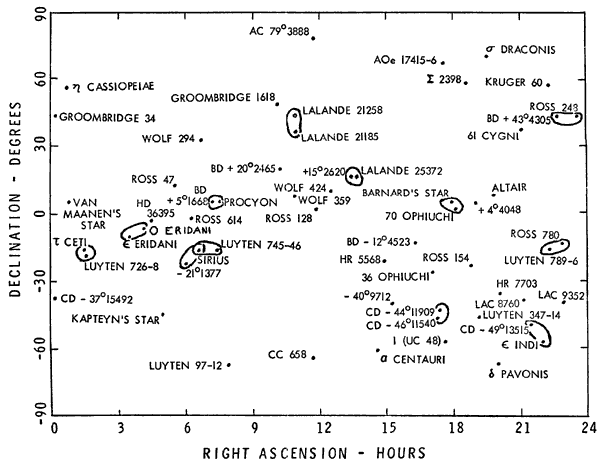
Of these Alpha Centauri is likely to be the focus of human hopes and expectations much as Mars has been. Mars is not only the closest planet, Venus excepted, it is the planet most like Earth. Alpha Centauri is the nearest star system; its two main components form a double star and both of them, by their luminosity and spectral characteristics, are found to be very like the sun.
There also are other stars similiar to the sun: Epsilon Eridani, Epsilon Indi, Tau Ceti, Omicron Eridani, 70 Ophiuchi, Eta Cassiopeiae, Sigma Draconis, 36 Ophiuchi, HR 7703, HR 5568, and Delta Pavonis. As is evident by their names, these are mostly the middle-of-the-road stars, not so brilliant as to be known by famous names, yet not so obscure as to merit only a designation from a star catalog.
In exploring these and others of interest, there will be recourse to a technique much appreciated at JPL. This is the technique of multiplanet flybys in which a spacecraft is sent past one planet on its way to the next. The Mariner Venus-Mercury mission of 1973-1975 was of this type, as is the 1973-1979 Pioneer 11 mission to Jupiter and Saturn. The years 1977 and 1978 will see the launching of two more missions of this type: another Jupiter-Saturn flight, and a three-planet mission to Jupiter, Saturn, and Uranus. In these flights the gravity of one planet changes the spacecraft direction and velocity to sling it on to the next.
Star-probe missions fly too rapidly to make use of the gravity even of stars to make course changes. But by reserving some propellant, it is possible for the starship to change its direction soon after passing the first star. It can visit two stars if the course change is not too large and can increase the scientific findings of a single mission.
A single flight can visit two very interesting stars: Barnard’s Star, which may have planets, and 70 Ophiuchi, a triple star one of whose components is very like the sun. En route to the potentially significant Tau Ceti, a star probe could detour and visit Luyten 726-8, a double star whose components are faint and reddish, and one of which emits strong flares. There could be a single mission to Epsilon Eridani and Omicron Eridani, and a flight to Epsilon Indi could then go on to the red dwarf CD — 49°13515. Sirius and Procyon would offer an additional inducement to visit them: their white-dwarf companions. These are highly compressed stellar remnants, in which something like the mass of the sun is contained in a volume only a few times that of Earth.
The ship would approach a white dwarf, gleaming balefully with its tiny disk. It would be pointed crosswise to its flight path to face the star and minimize heating and stress during the moments of closest approach. The white dwarf would loom up ahead, then flash past like some supernally brilliant light in a railway tunnel. In less than one second the ship would cross the whole of its surface and race on again into stellar space. The fierce gravity of the white dwarf would, in that brief encounter, so attract the starship that it would alter its course up to several degrees. If the trajectory were off and the starship hit the white dwarf, in its last milliseconds it would make some very interesting observations which would never be recorded. It would strike with the energy of a trillion tons of TNT, or 10,000 of the most powerful nuclear bombs ever detonated. Yet so vast are the energies released by stars, even by faint white dwarfs, that such an explosion would be little more than a candle in a forest fire. If a companion star probe happened to be observing the white dwarf at that exact moment, it might—might—see a momentary flicker in the star’s brightness.
These are the explorations and methods of discovery which will be carried into the next centuries. Such an ambitious long-term program of stellar exploration could only be carried out by the space colony. The colony would have both the resource base and the long-term stable future to carry it out and would have the industrial facilities to build the starships, those long and fragile creatures of zero g. The colony would recognize its long-term future prospects in the starships, for each night the colonists will face the stars, knowing them to be humanity’s future home.
What sort of findings from the star probes would most encourage the colonists? The most interesting discovery, of course, would be a planet like Earth—green with chlorophyll, blue with oceans, white with clouds. Yet even if such a planet is someday found, the discovery would not end but begin the needed explorations. There would be the problem of its indigenous life forms. Would they be harmful to us, causing pestilences or diseases, perhaps? Or would we prove harmful to them? Would we compete with them? Would we perhaps find that so fruitful and hopeful a garden-spot, so rare an oasis, was the property of someone else? The galaxy is very old—life, even intelligent life, may prove rather common and humanity is very young. It is far from inconceivable that within a time much shorter than has elapsed since Jesus preached in Galilee, we may be called to account for our stewardship of the planet systems closest to us.
There is little prospect of realizing the science-fiction scene of fast interstellar ships carrying small parties of explorers. When humans go to the stars, they will go in large groups and they will be prepared to stay. But how can they be sure they will find a life-sustaining home?
To answer this, let us remember again the dangers of planetary chauvinism, of arguing that only on a planet’s surface can people prosper. Let us remember the advantages of asteroids and other small celestial bodies in serving as a milieu for the growth of colonies. The answer is clear: in seeking to colonize the stars we should seek, not planets like Earth, but comets, small rocky bodies, including satellites of planets and asteroidlike debris left over from the origin of those stellar systems.
Collections of small bodies may be quite common. Alpha Centauri, a double star, may have no planets at all but each component may have extensive belts of asteroids which failed to form planets because of the gravitational influences of the other component. Collections such as these may be found and charted by even the earliest flyby missions, their surfaces studied and their compositions determined. The findings of the star probes can lead immediately to colonization efforts.
To colonize the stars, it will be necessary to build a small colony, along the lines of the Stanford torus, housing 1,000 people. Long experience with space colonies will have given our descendants an ample understanding of the problems in building closed-cycle systems in which there is neither leakage nor loss. To the colony will be fitted an array of starship engines together with an appropriate mass of propellant, surrounding it in an insulated structure to act as a radiation shield. With nuclear power for heat and light, the voyagers will live nearly as they and their forebears had always done ever since the dawn of space colonization.
If the target is Alpha Centauri or Barnard’s Star, the youngest members of the community will still be alive at the destination. More commonly the voyage will far exceed a human lifetime. They will drift through space—these tiny bright spores of life, these islands of hope in the blackness. The distant stars, like lights seen across a valley in winter, will offer no aid. The voyagers will be on their own.
When their descendants see one star glowing more brightly than the rest, when it shows a disk and becomes evident as the target, when the ship slows and at length ends its journey hard by some nameless asteroid—then the colonizing of a new star system will begin. Perhaps there will be a new Earth, a bright and blue habitable planet. Over the ensuing years, while the people work to recreate the colonies their ancestors had known, some will cautiously, tentatively approach it. They will send to it probes and orbiters, at length descending in small parties to its surface, and like Robinson Crusoe, perhaps they will find a footprint.
The star colonies will grow and prosper. They will communicate with Earth and each other, so star-flung humanity will not lose its common specieshood. In the thousands of years to come, new ships will set out to the star colonies. Nor will the people then forget their skills as spacefarers, for always the night sky will disclose further frontiers. There will be new starships, new voyages, originating not from our solar system but from other ones, and the history of the human reach outward will continue. In a few million years, in the time since the first pebble-tools, the whole of the galaxy may be the province of humanity. But long before then, we will quite likely have met other intelligent races far more advanced than we, and settled into our place in the community of interstellar civilizations.